PhD Insights: Making electric vehicles work for a better future
By Waseem Marzook, a member of the Transition to Zero Pollution cohort.
Electric vehicles (EVs) are key to achieving a carbon neutral and pollution free society. Transportation makes up a significant proportion of the global carbon footprint; one of the quickest and easiest way to greatly reduce that footprint is through the mass adoption of EVs, replacing all the fossil-fuel-powered vehicles on the road.
The most important component in any EV is the battery pack. Primarily powered by several lithium ion cells, EVs need long driving ranges, fast charging, and long warranties to compete with their fossil-fuel powered counterparts. This requires high capacity battery packs that are efficiently cooled and optimised for weight and cell lifetime.
Batteries age?
Unlike a petrol fuel tank, batteries age over time; the more they are used, and the more time that passes, the more their performance deteriorates. They store less charge, become more inefficient and deliver less peak power. For EVs this means that the maximum range and power are always reducing. Slowing the rate of this ageing, therefore, is a key component in improving EVs.
There are many factors that affect the rate of ageing such as current and the amount of charge in the battery. One of the biggest factors is temperature, extreme temperature both hot and cold have negative effects on battery life.
Keeping them cool
The key problem with fast charging is keeping the battery pack within a safe operating temperature. The battery pack generates a lot of heat while fast charging and this heat needs to be removed efficiently to keep all the cells at a safe and uniform temperature. Battery packs need to be cooled uniformly, as if you have one side of you pack in an optimal range but the other getting very hot, the hot side will age faster than the cold side. This can lead to premature failure of the entire pack.
Cell Cooling Coefficient
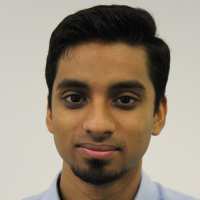
The Cell Cooling Coefficient (CCC) is a new universal measurement metric for characterising how efficiently a cell can be cooled. It tells you the temperature difference that will occur in a cell when a specified amount of heat is removed from it. My research involves developing this metric for cylindrical cells. Carefully designed rigs are used to experimentally measure the CCC of cylindrical cells of different sizes and under different cooling schemes, such as cooling the base of the cylinder or the sides. Longer term testing will show which cooling schemes are better at slowing down the ageing rate, coupling this with modelling of the CCC, this work will help identify where the thermal performance of these cells can be improved.
Cell manufacturers can use this metric optimise to their cells and produce the best thermally performing cell. As well as helping pack manufactures to compare a wide range of cells from different manufacturers, they will also be able to pick the best cell based on thermal performance for their cooling system. Ultimately, this will help develop battery packs that can be charged faster and which last longer. This will help mitigate some of the biggest downsides to electric vehicles and increase their desirability over fossil-fuel-powered vehicles, taking us a step closer to achieving a zero-pollution and carbon neutral planet.